the Creative Commons Attribution 4.0 License.
the Creative Commons Attribution 4.0 License.
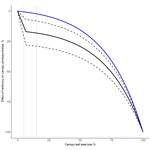
Low-intensity insect herbivory could have large effects on ecosystem productivity through reduced canopy photosynthesis
Kristiina Visakorpi
Sofia Gripenberg
Yadvinder Malhi
Terhi Riutta
Our current understanding of the effect of insect herbivory on ecosystem productivity is limited. Previous studies have typically quantified only the amount of leaf area loss or have been conducted during outbreak years when levels of herbivory are much higher than on average. These set-ups often do not take into account the physiological changes taking place in the remaining plant tissue after insect attack or do not represent typical, non-outbreak herbivore densities. Here, we estimate the effect of non-outbreak densities of insect herbivores on gross primary productivity in a temperate oak forest both through leaf area loss and through changes in leaf gas exchange. We first conduct a meta-analysis to assess evidence of herbivory-induced changes in photosynthesis in the literature. We then estimate how canopy primary productivity changes with decreasing and increasing levels of herbivory by using a canopy upscaling model and the average leaf-level effect based on the literature. The meta-analysis revealed a wide range of effects of herbivory on leaf photosynthesis, ranging from a reduction of 82 % to an increase of 49 %. On average, herbivory reduces the photosynthetic rate in the remaining leaf tissue by 16 % [6 %–27 %; 95 % CI]. The gross primary productivity of an oak stand under normal (5 % leaf area loss) levels of herbivory is estimated on average to be 13 % [5 %–21 %] lower than that of a non-herbivorized stand, once physiological changes in the intact plant tissue are considered. We propose that the effect of insect herbivory on primary productivity is non-linear and determined mainly by changes in leaf gas exchange and the pattern at which herbivory spreads through the canopy. We call for replicated studies in different systems to validate the relationship between insect herbivory and ecosystem productivity proposed here.
- Article
(1924 KB) - Full-text XML
- BibTeX
- EndNote
By affecting plant abundance, distribution, and physiology, herbivores can have large impacts on ecosystem carbon storage and cycling (Kurz et al., 2008; Schmitz et al., 2014). To date, many studies have demonstrated the role of large grazers and their predators in controlling primary productivity (Wilmers and Schmitz, 2016; Zimov et al., 2009) or insect outbreaks in causing large-scale defoliation and tree mortality (Clark et al., 2010; Edburg et al., 2012; Flower et al., 2013). Under outbreak densities, the effects of insect herbivores on forest carbon cycling can be large enough to shift the ecosystem from being a carbon sink into a carbon source (Heliasz et al., 2011; Lund et al., 2017). However, the role of non-outbreak, “background” densities of insect herbivores on carbon cycling has received less attention. The ubiquity of insect herbivory in terrestrial ecosystems and its profound effects on plant metabolism (Bilgin et al., 2010; Nabity et al., 2009; Nykänen and Koricheva, 2004) suggest that even low densities of insect herbivores could have a large impact on ecosystem carbon sequestration and loss.
Even low levels of insect herbivory can affect ecosystem carbon cycling through triggering physiological changes in the host plant. Insect herbivory has been shown to affect rates of photosynthesis beyond leaf area loss (“indirect effect of herbivory”, as opposed to “direct effect” of leaf area loss; Bilgin et al., 2010; Meza-Canales et al., 2017; Nabity et al., 2009; Zangerl et al., 2002). These effects can sometimes be large, for example, reducing photosynthesis by 80 % in the remaining leaf tissue (Delaney, 2012). The reduced photosynthesis has been linked to a shift to secondary metabolism (defence) at the expense of photosynthesis (Bilgin et al., 2010). Despite the evidence showing that insect herbivory can have large impacts on plant gas exchange, these physiological plant responses to herbivory have generally been neglected when estimating the impact of insect herbivores on ecosystem CO2 exchange. This might be because many of the studies reporting large herbivory-induced changes in plant gas exchange have been conducted in laboratory or greenhouse settings and on herbaceous species (Meza-Canales et al., 2017; Zangerl et al., 2002) or on potted seedlings (Kivimäenpää et al., 2016) and have focused on investigating the effects on small scales, for example, within leaves (Zangerl et al., 2002). Consequently, these effects are rarely taken into account in studies investigating ecosystem-scale carbon fluxes (but see Visakorpi et al., 2018), which are often carried out on woody species, over large geographic areas and using field-based measurements. As a result, we currently do not have a clear picture of how non-outbreak levels of insect herbivory affect ecosystem CO2 exchange. Ignoring potential effects of insect herbivory could lead to biased estimations of ecosystem productivity or of the role of forests as carbon sinks.
In this study, we estimate the effects of insect herbivory on primary productivity of a temperate forest canopy under varying levels of insect herbivory. We first search the literature on previously reported effects of insect herbivory on plant gas exchange of woody plants and use meta-analysis to scope how widespread and large these effects are likely to be. We then propose an approach for incorporating indirect effects of herbivory into canopy-level models on photosynthesis. We test how the effect of herbivory on canopy primary productivity depends on the amount of herbivory, the magnitude of indirect effect, and the pattern at which herbivory spreads through the canopy. Specifically, we ask the following:
-
Based on previous literature, how commonly reported are indirect effects of herbivory, and under what circumstances are they likely to be most pronounced?
-
If indirect effects are prevalent, what is the relationship between the intensity of herbivory and its impact on canopy photosynthesis?
2.1 Literature search
In October 2023, we searched on ISI Web of Science with the search string (herbivor* OR folivor*) AND (photosynth* OR “gas exchange”) AND (tree OR seedling OR wood* OR canopy) AND (experiment* OR treatment OR manipulati*). This yielded 270 articles, which were then screened by reading the abstract and, if needed, the methods. We included only studies on woody species (trees, shrubs, and seedlings), studies that had used manipulation of insect herbivory (excluding simulated herbivory, mammalian herbivory, and observational studies), and studies that had measured photosynthesis as gas exchange (excluding, for example, studies using chlorophyll fluorescence). In addition, we searched the reference lists of meta-analyses and the articles found directly through the search. Based on these criteria, we arrived at 31 papers. Information needed for study-specific effect size calculations (mean, standard deviation (SD), and sample size for each treatment group) was available for 26 of the papers, reporting data for a total of 51 experiments (Tables A1 and A2). Data for study-specific effect size calculations were extracted from the text, tables, and (if necessary) from figures. When a paper presented multiple treatments or experiments (different species, genotypes, herbivory levels, environmental conditions), we included the results from each treatment or experiment, yielding multiple effect size estimates for the effect of herbivory per study. When a paper presented data from one experiment but included repeated measurements of the same individuals or measurements from different parts of the plant (e.g. leaves), we averaged the photosynthetic measurements and estimated the sample size as the number of individuals per study. From repeated measurements over short time intervals (hours, days), we only extracted the last measurement point.
We used the extracted data to first estimate the standardized mean difference (SMD) in photosynthesis between the plants experiencing herbivory and the control plants for each experiment (Table A3). We estimated an overall effect size (Hedges' g) using a random-effects model with a restricted maximum likelihood estimator for the heterogeneity variance (τ2) and a Knapp–Hartung adjustment for estimating the confidence intervals. We then performed a subgroup analysis to test the effect of the following parameters on the magnitude of the SMD, performing a separate test for each parameter: plant clade (angiosperm vs. gymnosperm), plant growth form (e.g. seedling vs. tree), herbivore order, herbivore feeding guild, and type of measured leaf (intact vs. damaged). Testing for the significance between groups was done using a Q test, assuming separate estimates of the heterogeneity variance per group. We further tested the effect of host plant phylogeny by estimating mean SMD per host plant species and calculating phylogenetic signal statistics (Blomberg's K and K*, Abouheif's C mean, Moran's I, and Pagel's λ), which were tested for the null hypothesis of absence of signal in the magnitude of the indirect effect by randomization for K, K*, C mean, and I and by likelihood ratio test for λ (Keck et al., 2016). The host plant phylogeny was built by pruning a global mega-tree for vascular plants (Jin and Qian, 2019). To assess publication bias, we plotted the studies based on their effect size and error and investigated the resulting spread (“funnel plot”). We also performed Egger's regression test, which tests for a bias in meta-analysis results (Egger et al., 1997) using a corrected standard error formula proposed by Pustejovsky and Rodgers (2019).
For scaling up leaf-level measurements to a canopy level, an estimate of proportional change in photosynthesis is required, rather than the magnitude of the absolute mean difference between treatment and control. For this, we first estimated the proportional reduction or increase in photosynthesis due to herbivory for each study separately by dividing the difference in photosynthetic rate between control and treatment plants with the photosynthetic rate of the control plant (i.e. indirect effect of herbivory). We then calculated the overall mean and 95 % confidence interval (CI) of the indirect effect using the inverse variance method for pooling means across all the experiments. Because the canopy model we use describes a canopy photosynthesis of a broadleaf tree species, and because the subgroup analysis identified significant differences in indirect effect between plant clades (see Results), we pooled the indirect effects of studies on angiosperms only. This pooled estimate was then used in the subsequent canopy models (see below).
2.2 Simulating the effects of herbivory on canopy-level photosynthesis
To estimate how insect herbivory might affect the gross primary productivity of a tree canopy, we scaled up the estimated effects of herbivory from leaf-level measurements to a canopy scale. For this, we used the JULES big-leaf canopy model (Clark et al., 2011). In the big-leaf approach, light attenuates through the canopy following Beer's law (Monsi and Saeki, 1953), and photosynthesis is assumed to vary proportionally with changes in light intensity through the canopy. Leaf photosynthesis is thus expressed as a function of light-saturated photosynthetic rate (i.e. photosynthesis of leaves at the top of the canopy when exposed to full sunlight), leaf area index (i.e. the thickness of the canopy), and light extinction coefficient (describing how strongly the leaf tissue absorbs of reflects light). In addition, the model takes into account daytime respiration, which is assumed to be inhibited by increased light intensity (“Kok effect”; Kok, 1956), and is estimated using same principles. Daytime canopy net photosynthesis is then estimated as the integral of leaf-level photosynthesis over the entire canopy leaf area index (Clark et al., 2011). The big-leaf model is a relatively simple canopy model, which does not take into account for example direct and diffuse light through the multiple canopy layers. We chose to use this simple model, as we do not have the detailed information on light diffusion or leaf characteristics at different canopy layers that would be needed to benefit from a more complex model. Further, since our purpose is to describe the proportional change in canopy photosynthesis due to herbivory, using a model that takes into account the different canopy layers would be beneficial only with the information on how herbivory is distributed throughout different canopy layers, how decreasing leaf area due to herbivory affects the proportion of diffuse and direct light or sunflecks, or how rates of herbivory relate to or affect the distribution of leaf nitrogen concentration through the canopy. This information is currently lacking completely.
To estimate photosynthesis of a completely intact canopy, we used the following equation:
where P is canopy net photosynthesis (as of ground area), Asat is the light-saturated photosynthetic rate, k is a light extinction coefficient, L is the canopy leaf area index, l is the light intensity at the top of the canopy, K is the light intensity at which photosynthetic rate is half of its maximum, and Rd is the dark respiration rate estimated from the Michaelis–Menten equation. The light extinction coefficient (k) was set to 0.5 as a previously used estimate for broadleaf forests (Clark et al., 2011), and photosynthetically active radiation (PAR) was set to 1000 as a standard daytime light intensity at the top of the canopy.
We added the effect of herbivory to the equation as follows:
where the light-saturated photosynthetic rate is affected by the indirect change in photosynthesis (hi) in proportion to the amount of affected leaves in the canopy (p). Negative values of hi indicate reduced photosynthesis, whereas positive values indicate an increased (i.e. compensatory) photosynthesis. Further, herbivory affects the canopy-level photosynthesis by changing the leaf area index, which affects the overall canopy-level productivity (as reduced photosynthetic tissue), but also the amount of light available to lower canopy layers. This was captured by multiplying the leaf area index (L) with 1−d, where d is the direct effect, i.e. proportion of leaf area lost at the canopy scale. As some of the previous studies have shown no effect of herbivory on plant respiration rate (Aldea et al., 2005; Visakorpi et al., 2018), we assume here that respiration is not affected by herbivory (but see the Discussion section).
We used Eq. (2) to estimate how different levels of herbivory might affect canopy gross primary productivity. As an example study system, we used previously collected data from oak (Quercus robur) trees in Wytham Woods, (51°47′ N, 1°20′ W; 160 m a.s.l.), an ancient semi-natural woodland located in Oxfordshire, UK. We used previously measured values for the photosynthetic parameters (Asat, K, Rd) and leaf area index (L, Table 1; Fenn, 2010). Our previous work in this system (Visakorpi et al., 2018) has shown that photosynthesis of oak was substantially lower in leaves subjected to herbivory by winter moth (Operophtera brumata) caterpillars than in intact leaves surrounded only by other intact leaves. Moreover, a similar reduction in photosynthetic rate was seen in intact leaves on the same shoots as the damaged leaves, resulting in an estimated 50 % reduction in canopy-level photosynthesis. Our previous work was based on measurements of one herbivore species on 10 trees during two seasons with similar levels of herbivory and therefore represents only a snapshot of the effects insect herbivory can potentially have on ecosystem productivity. Here, we attempt to explore how the effect of herbivory on canopy gross primary productivity changes with changing herbivory pressure and magnitude of the indirect effect. To capture the likely range in the magnitude of the indirect effect of herbivory on photosynthesis, this parameter (hi) was set to vary ±95 % CI of the average effect based on the studies found through the literature survey.
2.3 Simulating how herbivory spreads across the canopy
Many studies on the indirect effects of herbivory have shown that the herbivory-induced changes in gas exchange can extend from the eaten leaf to the intact neighbouring leaves (Mäntylä et al., 2008; Visakorpi et al., 2018). For Eq. (2), we thus modelled the proportion of affected leaves (p) as a sum of leaves eaten by herbivores (pd) and intact leaves that are systemically affected (ps), i.e. p = pd + ps. The proportion of damaged leaves in the canopy (pd) was set to vary between 0 (no herbivory) and 1 (all leaves have signs of herbivory). We modelled two scenarios: (1) systemically affected leaves change their photosynthesis to the same extent as damaged leaves, or (2) systemically affected leaves do not change their photosynthesis (i.e. p = pd).
To simulate how increasing rates of herbivory affect the proportion of affected leaves and total canopy-level leaf area loss, we defined two parameters: the average leaf area loss per damaged leaf (hl) and the ratio of damaged leaves to systemically affected leaves (pd:ps). We assumed that hl and pd:ps stay at a constant level until all leaves are affected (either directly or systemically). For a conservative estimate, we set ps:pd = 0.5; in other words, for each damaged leaf, we assumed an area of a half a leaf which would be affected systemically. After all leaves are affected by herbivores, the proportion of damaged leaves was set to increase until all leaves were damaged to the extent of hl. Finally, we set hl to increase until the tree was completely defoliated. In other words, we assumed that the herbivory first spreads evenly to cover all intact, non-affected leaves, then from damaged leaves to systemically affected leaves, and finally increases per leaf. Whether this pattern holds depends on how quickly herbivores are likely to move to a new intact leaf after feeding on their current leaf (i.e. the parameter hl), which is likely affected by the feeding pattern of the specific herbivore species and how fast the host tree can start producing defences as a response to herbivory. We estimated three scenarios for hl: 10 % (close to the average leaf area loss per leaf found in field surveys; González-Zurdo et al., 2016; Kozlov, 2008; Visakorpi et al., 2018), 50 % (i.e. herbivores eat half of a leaf and then move on to the next leaf), and 1 % (i.e. herbivores make very little damage per leaf before moving on to feeding on intact leaves). The direct effect of herbivory (i.e. leaf area loss on the canopy scale, hd) is then a product of the proportion of damaged leaves (pd) and leaf area loss per damaged leaf (hl), i.e. d = pd⋅hl. See Table A4 for the summary of the different scenarios tested.
All analyses were carried out in R version 4.2.2 (R Core Team, 2022). Extraction of data from images was done with the package metaDigitise (Pick et al., 2019), and effect sizes were estimated with package meta (Balduzzi et al., 2019). Host plant phylogeny was built using packages V.PhyloMaker (Jin and Qian, 2019), phylobase (Hackathon, 2020), and phylosignal (Keck et al., 2016). The figures were created with the help of ggplot2 (Wickham, 2009), gridExtra (Auguie, 2017), and ggpubr (Kassambara, 2019).
3.1 Meta-analysis of data extracted from the literature
The studies reported results from 24 different plant and 23 different herbivore species. Most of the plant species were angiosperms (75 %). The herbivore species belonged to a variety of orders (33 % Lepidoptera, 25 % Coleoptera, 18 % Hymenoptera) and feeding guilds (57 % chewing, 20 % root-feeding, 16 % sap-sucking, and 6 % bark-feeding).
Most studies reported negative indirect effects of herbivory on photosynthetic rate (35 out of 51 experiments). The magnitude of the indirect effect ranged widely, from reduction of 82 % to an increase of 49 %. The standardized mean difference in photosynthetic rates was −0.52 [−0.93; −0.11, 95 % CI]. The proportion of the variance in study estimates due to heterogeneity between studies (I2, e.g. because of differences in experimental design, data analysis, or the treated population) was large (80.9 %). There were no significant differences in the magnitude of the indirect effect between different feeding guilds (Q = 1.75, df = 4, p = 0.78), herbivore orders (Q = 9.57, df = 4, p = 0.09), or plant growth forms (e.g. seedling vs. tree, Q = 1.78, df = 3, p = 0.62). The type of leaf measured in the herbivory treatment (damaged leaf vs. intact leaf from an otherwise damaged individual) had a marginally significant effect (Q = 5.29, df = 1, p = 0.07), suggesting that photosynthesis tends to be more strongly reduced when the measurements were taken from leaves showing signs of herbivore damage. The magnitude of indirect effect did differ between plant clades (Q = 8.71, df = 1, p = 0.003) being more pronounced (and negative) for angiosperms (Table A3). Host plant phylogeny had otherwise no effect (Fig. A1). The funnel plot (Fig. A2) and Egger's test (intercept = −1.58, SE = 0.65, t = −2.43, p = 0.02) suggested a negative bias in the meta-analysis results.
The average pooled indirect effect (i.e. proportional change in photosynthesis) was a reduction of 16 % [−27 %; −6 %, 95 % CI]. This value was further used to estimate the effect of herbivory on canopy-scale photosynthesis (see below).
3.2 Estimating effects of herbivory on canopy-scale photosynthesis
We estimate that under typical levels of herbivory (5 % leaf area loss on canopy scale; Cebrian, 2004), herbivory might reduce gross primary productivity (i.e. canopy-scale photosynthesis) by 13 % [21 %; 5 %, 95 % CI] (assuming hi of 16 %, i.e. the pooled indirect effect across studies). If indirect effects are missing (i.e. the effect of herbivory on primary productivity is through reduced leaf area alone), the same level of herbivory would result in only 0.5 % reduction in productivity.
When indirect effects are included in the model and if the average leaf area loss per damaged leaf (hl) is low, there is a clear step change in the effect of herbivory on canopy-level photosynthesis (Fig. 1). If indirect effects are missing or if the average leaf area loss per damaged leaf is 100 % (i.e. herbivores consume the whole leaf before moving to the next one), the step change disappears, and the relationship is continuous and exponential (Fig. 1). Changes in the extent of the systemic effect, i.e. whether the indirect effect of herbivory on photosynthesis spreads to the intact neighbouring leaves, have only a small influence on canopy-level photosynthesis or the shape of the relationship (Fig. A3).
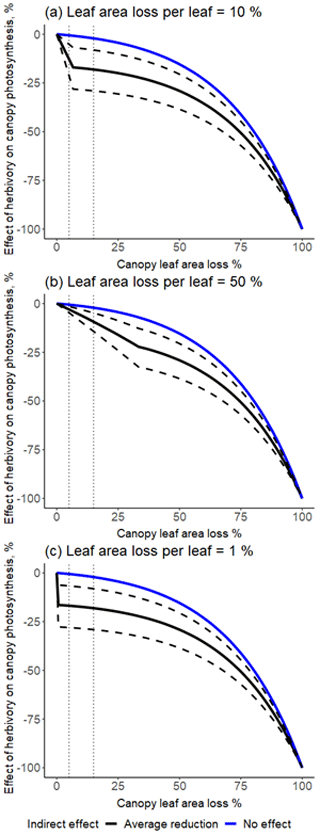
Figure 1The effect of herbivory on canopy-scale photosynthesis as a function of canopy-level leaf area loss, depending on the magnitude of the indirect effect (hi) and leaf area loss per damaged leaf (hl). The effect of herbivory on canopy photosynthesis was calculated as the proportional difference between a situation with no herbivory (Eq. 1) and one with herbivory included (Eq. 2), i.e. (. The blue line describes a scenario of no indirect effect (hi = 0), and the black line describes a scenario with the average indirect effect estimated from the literature (reduction of 16 %). The dashed black lines show the 95 % confidence intervals for the magnitude of the indirect effect for three different scenarios: average leaf area loss per damaged leaf (hl) under non-outbreak herbivore densities is 10 % (close to what is found in field surveys) (a), 50 % (hypothetically high) (b), and 1 % (hypothetically low) (c). The thin dotted vertical lines represent the lower and upper bounds of a typical level of herbivory in temperate forests (5 %–15 % of canopy-scale leaf area loss; Cebrian, 2004).
Ignoring existing indirect effects will result in an erroneous estimate of canopy-level gross primary productivity. If the indirect effect were large (21 %), ignoring it would result in a 20 %–28 % error in estimating canopy photosynthesis under typical herbivory levels (i.e. 5 %–15 % of leaf area loss on canopy scale).
In this study, we aim to evaluate the effect of typical levels of insect herbivory on canopy-scale gross primary productivity by taking into account both herbivory-induced changes in leaf gas exchange (indirect effects) and leaf area loss (direct effect). Our literature search and meta-analysis revealed several examples of indirect effects on woody species. The magnitude of the effect ranged considerably, though the majority of the studies reported a reduction in photosynthesis. When incorporating these effects into a canopy-scale model on photosynthesis, we estimate that canopy gross primary productivity can be reduced on average 13 % and up to 21 % even if leaf area loss is small (5 %). We suggest that the relationship between the intensity of herbivory and its effect on ecosystem productivity is non-linear and described by two stages: first, a rapid, linear increase in the effect of herbivory due to the spread of indirect effects in the canopy (suppression of photosynthesis) and, second, a slower non-linear increase with increasing leaf area loss. We identify two parameters that are important in shaping the relationship between leaf area loss and change in canopy primary productivity: the magnitude of indirect effect (hi) determines the magnitude of change in productivity, and average leaf area loss per damaged leaf (hl) determines the shape of the relationship. Below, we discuss our findings and suggest ways forward for clarifying the role insect herbivores can have on ecosystem productivity.
4.1 The potential effect of insect herbivory on ecosystem productivity
We suggest that insect herbivores can have a large impact on forest primary productivity even at a low density. These “background” levels of insect herbivory have received little attention in the literature to date. Instead, many previous studies on the effects of insect herbivores on ecosystem productivity have concentrated on outbreak densities of insects, reporting considerable losses of carbon from the ecosystem due to large-scale defoliation and tree mortality (Heliasz et al., 2011; Kurz et al., 2008; Lund et al., 2017; Schäfer et al., 2010). For example, a gypsy moth outbreak in an oak–pine forest reduced canopy photosynthesis by 24 % (Schäfer et al., 2010), and moth outbreak in a mountain birch forest reduced the strength of the ecosystem carbon sink by 89 % (Heliasz et al., 2011). In contrast, we estimate a potential reduction of 13 % in canopy-scale photosynthesis from just 5 % of leaf area loss. The gross primary productivity of the oak forest we used as an example study system has been estimated to be between 21.1 Mg C ha−1 yr−1 (Thomas et al., 2011) and 21.9 Mg C ha−1 yr−1 (Fenn et al., 2015). This means that 1.1–4.6 Mg C ha−1 yr−1 of gross primary productivity could be lost to herbivory even under very low levels of leaf area loss. In contrast, assuming average carbon content (47.1 %) and leaf mass per area (63.2 g m−2) of an oak leaf (Visakorpi et al., 2020), the carbon lost to herbivores through direct leaf area loss of 5 % is only 0.097 Mg C ha−1 yr−1. The “invisible” effect of altered photosynthesis can therefore have a much greater influence on ecosystem carbon balance than the more visible leaf area loss. Nevertheless, it is important to emphasize that the results we present are influenced by the modelling approach we have chosen and the specific parameters included. We call for replicate field studies to better understand the magnitude of the effects in natural settings.
A handful of previous studies support our suggestion that low-intensity insect herbivory can be an important driver of ecosystem-level processes (e.g. see Metcalfe et al., 2014). For example, a small increase in low-intensity herbivory (from 1 % to 3 % leaf area loss) in mountain birch (Betula pubescens) resulted in a much larger (30 %) reduction in plant growth (Zvereva et al., 2012). Similarly, a suppression of herbivory from 4 % to 1 % doubled the biomass production in a boreal forest (Shestakov et al., 2020). In a mesocosm experiment, the presence of predators led to a lower consumption of plants by herbivores, resulting in up to a 1.4-fold increase in carbon retained in the plant biomass, despite no significant difference in overall plant biomass (Strickland et al., 2013). Together, these studies suggest that the effect of herbivory on the ecosystem carbon cycle might be mediated mostly by the indirect effects of herbivory.
4.2 The relationship between intensity of herbivory and its effect on ecosystem productivity
Our model suggests that the effect of herbivory on canopy primary productivity with increasing levels of herbivores is non-linear, with two distinct stages. First, as the level of herbivory increases, the proportion of intact leaves decreases until all leaves are affected by herbivory (either directly or through systemic effects). Canopy-level primary productivity changes quickly and linearly during this phase. After the point at which all leaves are affected, further changes in canopy photosynthesis are caused entirely by the direct effects of leaf area loss, which increases exponentially.
The change between these two phases is unlikely to be as sharp in actual ecosystems as we predict. Importantly, we identify that the shape of this relationship likely depends on how herbivores move through the canopy, especially how easily they move to feed on new, yet intact, leaf tissue. This value is likely different for different ecosystems and plant and herbivore species and could potentially depend on how fast the host plants trigger the production of induced defences in the eaten leaf (Edwards and Wratten, 1983).
Supporting our suggestion of a non-linear relationship, Flower and Gonzalez-Meler (2015) showed in a meta-analysis how the relationship between pest outbreak intensity and its effect on forest net primary productivity was sigmoidal rather than linear, though the effects of herbivores were visible after at least 30 % of the basal area was affected. More studies including different study systems and species are needed to validate whether the proposed relationship between intensity of herbivory and its effect on plant productivity holds. Where there is strong interannual variability in herbivory, ecosystem measurements of productivity, such as eddy covariance approaches, or detailed estimates on tree growth could be coupled with herbivory measurements to test this relationship.
4.3 Existing knowledge gaps
In addition to the knowledge gaps identified above, there are several other aspects of how insect herbivory affects plant physiology and ecosystem-level processes that would need to be studied further. Despite our efforts to search the previous literature, it remains unresolved how common the indirect effects of herbivory truly are. This is difficult to assess from the literature alone, as non-significant results from such studies are less likely to be published. Our analysis revealed potential bias in the published results, though it is difficult to say whether the bias is due to publication bias (i.e. some studies are less likely published) or because of a large variation in results between the studies. The between-study heterogeneity was indeed large (81 %), and the indirect effect seems to vary considerably between different experiments. Nevertheless, our subgroup analysis was not able to detect any other significant parameters affecting the magnitude of the indirect effect other than plant clade (the effect being more negative for angiosperms), which is likely due to a low overall number of studies on the topic. Ideally, how common and large indirect effects of herbivory are should be tested experimentally on several tree species in field settings.
There are also likely to be several compensatory factors that might influence how herbivory affects ecosystem productivity which have not been considered in our predictions. First, the reduced leaf area caused by herbivory would increase light penetration to lower canopy layers (Anten and Ackerly, 2001) and increase canopy light use efficiency (Gough et al., 2013), to a larger extent than captured in our canopy model. Even though our model accounts for light diffusion through the canopy and our simulations assume that leaf area index changes with herbivory, a more detailed canopy model taking into account sunlit and shaded areas could simulate the effects of increased light more accurately. Second, late-season leaf flushes (e.g. “lammas shoots” on oak) might compensate for lost photosynthesis earlier during the growing season (Clark et al., 2010), especially if more leaves are produced as a response to herbivory. Third, increased herbivory is likely to also result in increased deposits of frass and leaf fragments and nutrient leaching from damaged leaves. The increased nutrient cycling and interception of light through the canopy could increase forest productivity and compensate for the loss of carbon to herbivory on an ecosystem scale (Costilow et al., 2017; Gough et al., 2013; Lund et al., 2017). Finally, insect feeding can increase transport of photosynthetic end products away from certain leaves and thereby reduce the negative effects of herbivory on photosynthesis (Retuerto et al., 2004; Schwachtje and Baldwin, 2008). These types of sink–source dynamics might change the distribution of photosynthetic efficiency within the canopy, resulting in higher photosynthetic rates in other parts of the canopy. In general, compensatory processes might explain why some models and flux tower measurements have failed to notice effects of insect defoliation beyond the impact of leaf area loss (Cook et al., 2008).
Herbivory is likely to also affect other aspects of plant metabolism, especially respiration rate, though the effects of herbivory on leaf, stem, or root respiration remain largely unknown. Herbivory can increase plant respiration (Cook et al., 2008; Strickland et al., 2013), for example, as a response to repair of damaged tissue, or respiration might follow photosynthetic rate and either increase or decrease to a similar extent (Whitehead et al., 2004). Increased leaf herbivory could increase carbon allocation to roots (Dyer et al., 1991), which might increase root respiration (Holland et al., 1996). If respiration were significantly increased by herbivory, the overall net primary productivity of the canopy would be impacted even more than in the case where only photosynthesis is reduced.
We suggest that insect herbivores, even at low densities, might have a considerable effect on ecosystem primary productivity through indirect changes in the rates of canopy gas exchange. If this is the case, forest ecosystems might naturally be in a state where photosynthesis is reduced by herbivory. The relationship between the intensity of herbivory and its effects on the host plant is likely non-linear. At typical herbivore densities (∼ 5 % leaf area loss), the effect of herbivory on canopy photosynthesis is primarily driven by changes in leaf gas exchange in the remaining leaf tissue, while the contribution of leaf area loss increases with increasing herbivore abundances. We predict that comparisons of primary productivity between completely intact plants and plants with even a small amount of herbivory-inflicted damage could yield large estimates on the effects of herbivory on plant productivity. On the other hand, comparisons between plants experiencing low (but non-zero) and high levels of herbivory might result in lower estimates on the effect of herbivory. The distributions and abundances of insect herbivores are likely to change in the future (Ayres and Lombardero, 2000; Kurz et al., 2008), with increasing temperature (Bale et al., 2002), CO2 concentration (Stiling et al., 2009), drought (Gaylord et al., 2013), and advancing phenology (Charmantier et al., 2008) affecting the intensity of herbivory differently. Quantifying the effect of insect herbivores on carbon cycling is therefore an intriguing and important avenue of research. We call for replicate field studies on the effects of insect herbivory on ecosystem productivity to validate whether the model-based results hold in natural settings.
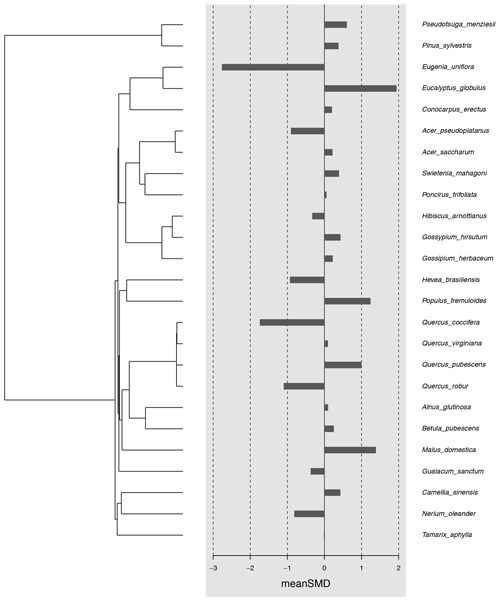
Figure A1The magnitude of the indirect effect based on the host plant phylogeny. The black bars correspond to the standardized mean difference per species estimated based on the meta-analysis. Host plant phylogenetic tree is shown to the left and host plant name to the right. The significance of the phylogenetic effect was tested by estimating different phylogenetic signal metrics (C mean = −0.34, p = 0.99; I = −0.09, p = 0.92; K = 0.15, p = 0.86, K* = 0.20, p = 0.85; λ < 0.001, p = 1).
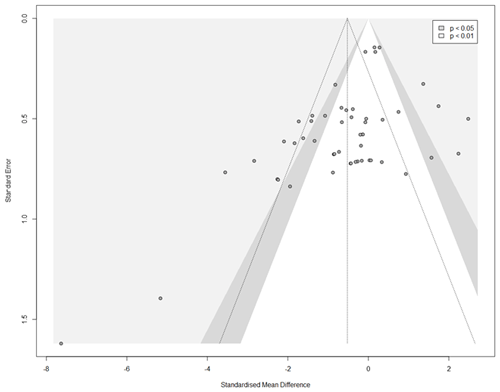
Figure A2Funnel plot for estimating publication bias in the meta-analysis. The grey dots are the 51 experiments included in the meta-analysis (see Table A1), plotted by their effect size (SMD) and standard error. Studies that fall into the grey-shaded areas report significant (p < 0.05 for dark grey, p < 0.01 for light grey) results. The triangle of dotted lines shows the expected distribution of studies without any publication bias (i.e. reported effect sizes closer to the estimated mean in studies with smaller error).
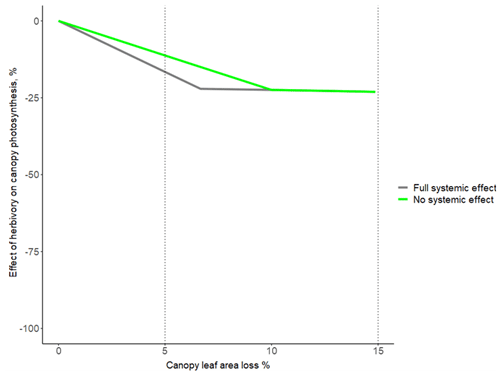
Figure A3The influence of the extent of systemic effect on how herbivory affects canopy photosynthesis, shown for the range 0 %–15 % canopy leaf area loss. The grey line corresponds to the black line in Fig. 1a (hi = 16 % and hl = 10 %), and systemically affected leaves (i.e. intact leaves neighbouring damaged leaves) reduce their photosynthesis to the same extent as damaged leaves. The green line corresponds to a situation where systemic effects are missing (i.e. only damaged leaves reduce their photosynthesis).
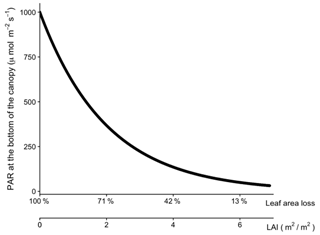
Figure A4The relationship between leaf area index (L), leaf area loss, and the amount of light at the bottom of the canopy when the light intensity is 1000 PAR at the top.
Table A1Overview of the studies from the literature survey included in the meta-analysis. Results from the literature survey on the extent of indirect effects of herbivory on the photosynthetic rate of woody plants. Shown are studies from which it was possible to extract data for the meta-analysis. Plant clade: A – angiosperm, G – gymnosperm. Indirect effect is reported as percent reduction or increase in photosynthetic rate in treated plant or leaf compared to the control. “Leaf type” identifies whether the measurements were taken from a damaged plant part or from an intact leaf from an otherwise damaged individual. If a study reported several experiments on the same plant–herbivore species pair, these are identified in the “Experiment” column. All manipulations are additions of herbivory, expect Wagner et al. (2020) and Lei and Wilson (2004), in which the manipulation was removal of herbivory by insecticide. The table is ordered by increasing magnitude of the indirect effect.
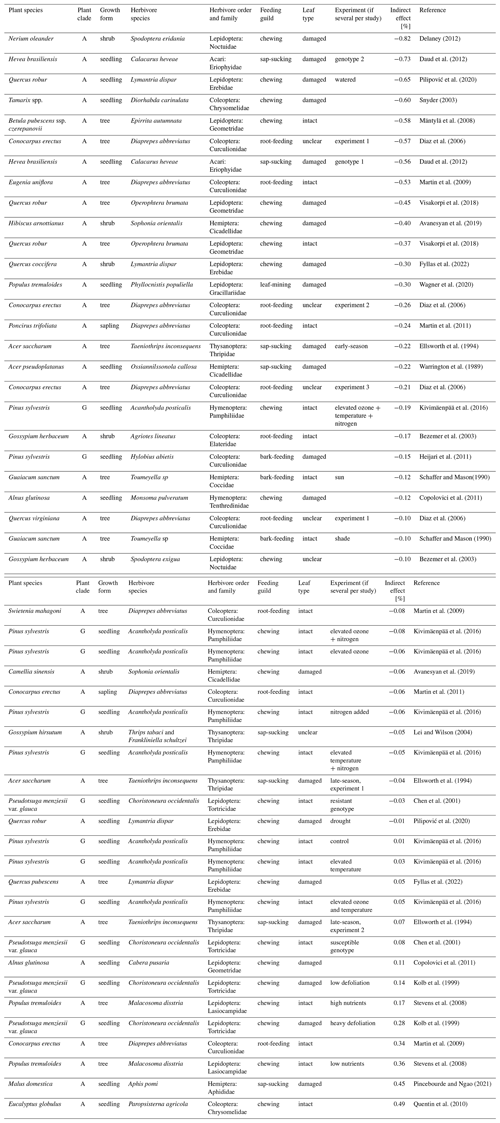
Table A2The results from the meta-analysis, shown here for the standardized mean difference in photosynthesis between control plants and plants experiencing herbivory, separately for angiosperms and gymnosperms. “Experimental” mean, SD, and total refer to the mean photosynthetic rate, standard deviation, and sample size of leaves in the herbivory treatment, and “Control” refers to the leaves or plants that have been kept intact in the experiments. “Weight” refers to how much the results from that particular study influence the overall effect size. “Standard mean difference” and the corresponding 95 % confidence intervals (CI) refer to the absolute difference in photosynthetic rates between intact plants and plants experiencing herbivory. Negative values mean a reduction in photosynthesis due to herbivory. Green boxes present the standardized mean difference (SMD) per study with 95 % CI. The black diamond at the bottom of the graph shows the mean pooled SMD.
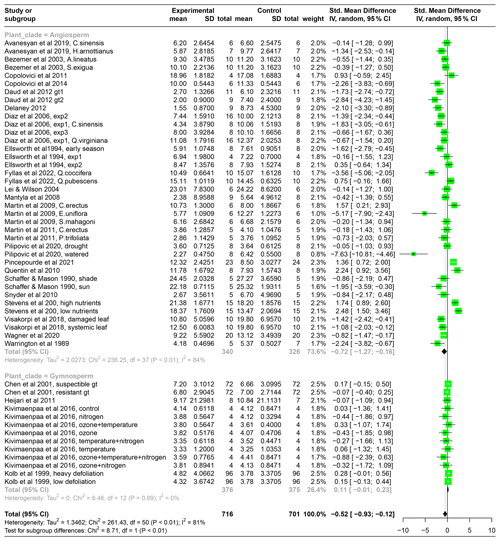
Table A3Results from subgroup analysis: “k” is the number of experiments per subgroup, SDM refers to the standardized mean difference in that subgroup, and the 95 % CIs are the confidence intervals for that mean difference. τ2 describes the between-study heterogeneity variance within subgroups. I2 refers to the heterogeneity in results within that subgroup.
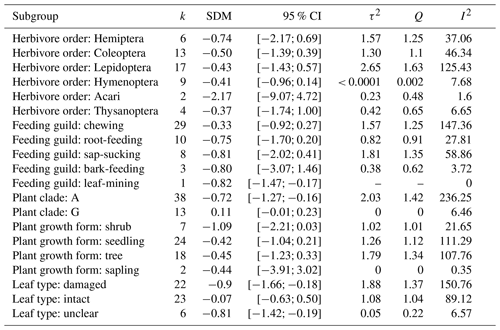
Table A4Summary of the different herbivory scenarios tested. To investigate the relationship between the intensity of insect herbivory and its effect on canopy-scale photosynthesis, we simulated different scenarios by changing the values of the model parameters. The table below summarizes the different scenarios tested and the corresponding results.
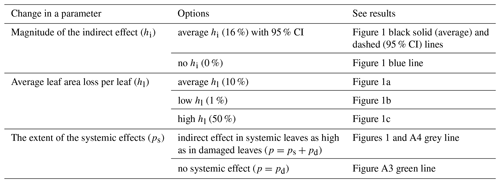
The data to perform the meta-analysis and R code to reproduce the analyses are available on Zenodo at https://doi.org/10.5281/zenodo.13886133 (Visakorpi, 2024).
KV, SG, YM, and TR came up with and developed the idea. KV carried out the literature search and modelling and wrote the first version of the manuscript. All authors contributed substantially to the subsequent versions.
The contact author has declared that none of the authors has any competing interests.
Publisher’s note: Copernicus Publications remains neutral with regard to jurisdictional claims made in the text, published maps, institutional affiliations, or any other geographical representation in this paper. While Copernicus Publications makes every effort to include appropriate place names, the final responsibility lies with the authors.
We thank the Journal Club of the Ecosystems lab and the reviewers for helpful comments on the text.
This research has been supported by Osk. Huttusen säätiö, Suomen Kulttuurirahasto, the Jackson Foundation, and the European Research Council, Horizon Europe European Research Council (grant no. GEM-TRAIT: 321131).
This paper was edited by Roland Brandl and reviewed by two anonymous referees.
Aldea, M., Hamilton, J. G., Resti, J. P., Zangerl, A. R., Berenbaum, M. R., and DeLucia, E. H.: Indirect effects of insect herbivory on leaf gas exchange in soybean, Plant Cell Environ., 28, 402–411, https://doi.org/10.1111/j.1365-3040.2005.01279.x, 2005.
Anten, N. P. R. and Ackerly, D. D.: Canopy-level photosynthetic compensation after defoliation in a tropical understorey palm, Funct. Ecol., 15, 252–262, 2001.
Auguie, B.: gridExtra: Miscellaneous Functions for “Grid” Graphics, Version 2.3, CRAN, https://doi.org/10.32614/CRAN.package.gridExtra, 2017.
Avanesyan, A., Snook, K. A., Follett, P. A., and Lamp, W. O.: Short-term physiological response of a native Hawaiian plant, Hibiscus arnottianus, to injury by the exotic leafhopper, Sophonia orientalis (Hemiptera: Cicadellidae), Environ. Entomol., 48, 363–369, https://doi.org/10.1093/ee/nvy193, 2019.
Ayres, M. P. and Lombardero, M. J.: Assessing the consequences of global change for forest disturbance from herbivores and pathogens, Sci. Total Environ., 262, 263–286, https://doi.org/10.1016/S0048-9697(00)00528-3, 2000.
Balduzzi, S., Rücker, G., and Schwarzer, G.: How to perform a meta-analysis with R: a practical tutorial, BMJ Ment. Health, 22, 153–160, https://doi.org/10.1136/ebmental-2019-300117, 2019.
Bale, J. S., Masters, G. J., Hodkinson, I. D., Awmack, C., Bezemer, T. M., Brown, V. K., Butterfield, J., Buse, A., Coulson, J. C., Farrar, J., Good, J. E. G., Harrington, R., Hartley, S., Jones, T. H., Lindroth, R. L., Press, M. C., Symrnioudis, I., Watt, A. D., and Whittaker, J. B.: Herbivory in global climate change research: direct effects of rising temperature on insect herbivores, Glob. Change Biol., 8, 1–16, https://doi.org/10.1046/j.1365-2486.2002.00451.x, 2002.
Bezemer, T. M., Wagenaar, R., Van Dam, N. M., and Wäckers, F. L.: Interactions between above- and belowground insect herbivores as mediated by the plant defense system, Oikos, 101, 555–562, https://doi.org/10.1034/j.1600-0706.2003.12424.x, 2003.
Bilgin, D. D., Zavala, J. A., Zhu, J., Clough, S. J., Ort, D. R., and DeLucia, E. H.: Biotic stress globally downregulates photosynthesis genes, Plant Cell Environ., 33, 1597–1613, https://doi.org/10.1111/j.1365-3040.2010.02167.x, 2010.
Cebrian, J.: Role of first-order consumers in ecosystem carbon flow, Ecol. Lett., 7, 232–240, https://doi.org/10.1111/j.1461-0248.2004.00574.x, 2004.
Charmantier, A., McCleery, R. H., Cole, L. R., Perrins, C., Kruuk, L. E. B., and Sheldon, B. C.: Adaptive phenotypic plasticity in response to climate change in a wild bird population, Science, 320, 800–803, https://doi.org/10.1126/science.1157174, 2008.
Chen, Z., Kolb, T. E., and Clancy, K. M.: Mechanisms of Douglas-fir resistance to western spruce budworm defoliation: bud burst phenology, photosynthetic compensation and growth rate, Tree Physiol., 21, 1159–1169, https://doi.org/10.1093/treephys/21.16.1159, 2001.
Clark, D. B., Mercado, L. M., Sitch, S., Jones, C. D., Gedney, N., Best, M. Clark, D. B., Mercado, L. M., Sitch, S., Jones, C. D., Gedney, N., Best, M. J., Pryor, M., Rooney, G. G., Essery, R. L. H., Blyth, E., Boucher, O., Harding, R. J., Huntingford, C., and Cox, P. M.: The Joint UK Land Environment Simulator (JULES), model description – Part 2: Carbon fluxes and vegetation dynamics, Geosci. Model Dev., 4, 701–722, https://doi.org/10.5194/gmd-4-701-2011, 2011.
Clark, K. L., Skowronski, N., and Hom, J.: Invasive insects impact forest carbon dynamics: defoliation and forest carbon dynamics, Glob. Change Biol., 16, 88–101, https://doi.org/10.1111/j.1365-2486.2009.01983.x, 2010.
Cook, B. D., Bolstad, P. V., Martin, J. G., Heinsch, F. A., Davis, K. J., Wang, W., Desai, A. R., and Teclaw, R. M.: Using light-use and production efficiency models to predict photosynthesis and net carbon exchange during forest canopy disturbance, Ecosystems, 11, 26–44, https://doi.org/10.1007/s10021-007-9105-0, 2008.
Copolovici, L., Kännaste, A., Remmel, T., Vislap, V., and Niinemets, Ü.: Volatile emissions from Alnus glutionosa induced by herbivory are quantitatively related to the extent of damage, J. Chem. Ecol., 37, 18–28, https://doi.org/10.1007/s10886-010-9897-9, 2011.
Costilow, K. C., Knight, K. S., and Flower, C. E.: Disturbance severity and canopy position control the radial growth response of maple trees (Acer spp.) in forests of northwest Ohio impacted by emerald ash borer (Agrilus planipennis), Ann. Forest Sci., 74, 10, https://doi.org/10.1007/s13595-016-0602-1, 2017.
Daud, R. D., De Cássia Conforto, E., and Feres, R. J. F.: Changes in leaf physiology caused by Calacarus heveae (Acari, Eriophyidae) on rubber tree, Exp. Appl. Acarol., 57, 127–137, https://doi.org/10.1007/s10493-012-9552-y, 2012.
Delaney, K. J.: Nerium oleander indirect leaf photosynthesis and light harvesting reductions after clipping injury or Spodoptera eridania herbivory: High sensitivity to injury, Plant Sci., 185–186, 218–226, https://doi.org/10.1016/j.plantsci.2011.10.012, 2012.
Diaz, A. P., Mannion, C., and Schaffer, B.: Effect of root feeding by Diaprepes abbreviatus (Coleoptera: Curculionidae) larvae on leaf gas exchange and growth of three ornamental tree species, J. Econ. Entomol., 99, 811–821, https://doi.org/10.1093/jee/99.3.811, 2006.
Dyer, M. I., Acra, M. A., Wang, G. M., Coleman, D. C., Freckman, D. W., McNaughton, S. J., and Strain, B. R.: Source-sink carbon relations in two Panicum coloratum ecotypes in response to herbivory, Ecology, 72, 1472–1483, https://doi.org/10.2307/1941120, 1991.
Edburg, S. L., Hicke, J. A., Brooks, P. D., Pendall, E. G., Ewers, B. E., Norton, U., Gochis, D., Gutmann, E. D., and Meddens, A. J.: Cascading impacts of bark beetle-caused tree mortality on coupled biogeophysical and biogeochemical processes, Front. Ecol. Environ., 10, 416–424, https://doi.org/10.1890/110173, 2012.
Edwards, P. J. and Wratten, S. D.: Wound induced defences in plants and their consequences for patterns of insect grazing, Oecologia, 59, 88–93, https://doi.org/10.1007/BF00388079, 1983.
Egger, M., Smith, G. D., Schneider, M., and Minder, C.: Bias in meta-analysis detected by a simple, graphical test, BMJ, 315, 629–634, https://doi.org/10.1136/bmj.315.7109.629, 1997.
Ellsworth, D. S., Tyree, M. T., Parker, B. L., and Skinner, M.: Photosynthesis and water-use efficiency of sugar maple (Acer saccharum) in relation to pear thrips defoliation, Tree Physiol., 14, 619–632, https://doi.org/10.1093/treephys/14.6.619, 1994.
Fenn, K., Malhi, Y., Morecroft, M., Lloyd, C., and Thomas, M.: The carbon cycle of a maritime ancient temperate broadleaved woodland at seasonal and annual scales, Ecosystems, 18, 1–15, https://doi.org/10.1007/s10021-014-9793-1, 2015.
Fenn, K. M.: Carbon cycling in British deciduous woodland: processes, budgets, climate & phenology, DPhil, University of Oxford, 2010.
Flower, C. E. and Gonzalez-Meler, M. A.: Responses of temperate forest productivity to insect and pathogen disturbances, Annu. Rev. Plant Biol., 66, 547–569, https://doi.org/10.1146/annurev-arplant-043014-115540, 2015.
Flower, C. E., Knight, K. S., and Gonzalez-Meler, M. A.: Impacts of the emerald ash borer (Agrilus planipennis Fairmaire) induced ash (Fraxinus spp.) mortality on forest carbon cycling and successional dynamics in the eastern United States, Biol. Invasions, 15, 931–944, https://doi.org/10.1007/s10530-012-0341-7, 2013.
Fyllas, N. M., Chrysafi, D., Avtzis, D. N., and Moreira, X.: Photosynthetic and defensive responses of two Mediterranean oaks to insect leaf herbivory, Tree Physiol., 42, 2282–2293, https://doi.org/10.1093/treephys/tpac067, 2022.
Gaylord, M. L., Kolb, T. E., Pockman, W. T., Plaut, J. A., Yepez, E. A., Macalady, A. K., Pangle, R. E., and McDowell, N. G.: Drought predisposes piñon-juniper woodlands to insect attacks and mortality, New Phytol., 198, 567–578, https://doi.org/10.1111/nph.12174, 2013.
González-Zurdo, P., Escudero, A., Nuñez, R., and Mediavilla, S.: Losses of leaf area owing to herbivory and early senescence in three tree species along a winter temperature gradient, Int. J. Biometeorol., 60, 1661–1674, https://doi.org/10.1007/s00484-016-1156-5, 2016.
Gough, C. M., Hardiman, B. S., Nave, L. E., Bohrer, G., Maurer, K. D., Vogel, C. S., Nadelhoffer, K. J., and Curtis, P. S.: Sustained carbon uptake and storage following moderate disturbance in a Great Lakes forest, Ecol. Appl., 23, 1202–1215, https://doi.org/10.1890/12-1554.1, 2013.
Hackathon, R.: phylobase: base package for phylogenetic structures and comparative data, Version 0.8.12, CRAN, https://doi.org/10.32614/CRAN.package.phylobase, 2020.
Heijari, J., Blande, J. D., and Holopainen, J. K.: Feeding of large pine weevil on Scots pine stem triggers localised bark and systemic shoot emission of volatile organic compounds, Environ. Exp. Bot., 71, 390–398, https://doi.org/10.1016/j.envexpbot.2011.02.008, 2011.
Heliasz, M., Johansson, T., Lindroth, A., Mölder, M., Mastepanov, M., Friborg, T., Callaghan, T. V., and Christensen, T. R.: Quantification of C uptake in subarctic birch forest after setback by an extreme insect outbreak: carbon uptake setback by insect outbreak, Geophys. Res. Lett., 38, L01704, https://doi.org/10.1029/2010GL044733, 2011.
Holland, J. N., Cheng, W., and Crossley, D. A.: Herbivore-induced changes in plant carbon allocation: assessment of below-ground C fluxes using carbon-14, Oecologia, 107, 87–94, https://doi.org/10.1007/BF00582238, 1996.
Jin, Y. and Qian, H.: V.PhyloMaker: an R package that can generate very large phylogenies for vascular plants, Ecography, 42, 1353–1359, https://doi.org/10.1111/ecog.04434, 2019.
Kassambara, A.: ggpubr: 'ggplot2' Based Publication Ready Plots, Version 0.6.0, CRAN, https://doi.org/10.32614/CRAN.package.ggpubr, 2019.
Keck, F., Rimet, F., Bouchez, A., and Franc, A.: phylosignal: an R package to measure, test, and explore the phylogenetic signal, Ecol. Evol., 6, 2774–2780, https://doi.org/10.1002/ece3.2051, 2016.
Kivimäenpää, M., Ghimire, R. P., Sutinen, S., Häikiö, E., Kasurinen, A., Holopainen, T., and Holopainen, J. K.: Increases in volatile organic compound emissions of Scots pine in response to elevated ozone and warming are modified by herbivory and soil nitrogen availability, Eur. J. For. Res., 135, 343–360, https://doi.org/10.1007/s10342-016-0939-x, 2016.
Kok, B.: On the inhibition of photosynthesis by intense light, Biochim. Biophys. Acta, 21, 234–244, https://doi.org/10.1016/0006-3002(56)90003-8, 1956.
Kolb, T. E., Dodds, K. A., and Clancy, K. M.: Effect of Western spruce budworm defoliation on the physiology and growth of potted Douglas-fir seedlings, Forest Sci., 45, 280–291, https://doi.org/10.1093/forestscience/45.2.280, 1999.
Kozlov, M. V.: Losses of birch foliage due to insect herbivory along geographical gradients in Europe: a climate-driven pattern?, Climatic Change, 87, 107–117, https://doi.org/10.1007/s10584-007-9348-y, 2008.
Kurz, W. A., Dymond, C. C., Stinson, G., Rampley, G. J., Neilson, E. T., Carroll, A. L., Ebata, T., and Safranyik, L.: Mountain pine beetle and forest carbon feedback to climate change, Nature, 452, 987–990, https://doi.org/10.1038/nature06777, 2008.
Lei, T. T. and Wilson, L. J.: Recovery of leaf area through accelerated shoot ontogeny in thrips-damaged cotton seedlings, Annals of Botany, 94, 179–186, https://doi.org/10.1093/aob/mch125, 2004.
Lund, M., Raundrup, K., Westergaard-Nielsen, A., López-Blanco, E., Nymand, J., and Aastrup, P.: Larval outbreaks in West Greenland: Instant and subsequent effects on tundra ecosystem productivity and CO2 exchange, Ambio, 46, 26–38, https://doi.org/10.1007/s13280-016-0863-9, 2017.
Mäntylä, E., Alessio, G. A., Blande, J. D., Heijari, J., Holopainen, J. K., Laaksonen, T., Piirtola, P., and Klemola, T.: From plants to birds: higher avian predation rates in trees responding to insect herbivory, PLoS ONE, 3, e2832, https://doi.org/10.1371/journal.pone.0002832, 2008.
Martin, C. G., Mannion, C., and Schaffer, B.: Effects of herbivory by Diaprepes abbreviatus (Coleoptera: Curculionidae) larvae on four woody ornamental plant species, J. Econ. Entomol., 102, 1141–1150, https://doi.org/10.1603/029.102.0336, 2009.
Martin, C. G., Mannion, C., and Schaffer, B.: Leaf gas exchange and growth responses of Green Buttonwood and Swingle Citrumelo to Diaprepes abbreviatus (Coleoptera: Curculionidae) larval feeding and flooding, Fla. Entomol., 94, 279–289, https://doi.org/10.1653/024.094.0222, 2011.
Metcalfe, D. B., Asner, G. P., Martin, R. E., Silva Espejo, J. E., Huasco, W. H., Farfán Amézquita, F. F., Carranza-Jimenez, L., Galiano Cabrera, D. F., Baca, L. D., Sinca, F., Huaraca Quispe, L. P., Taype, I. A., Mora, L. E., Dávila, A. R., Solórzano, M. M., Puma Vilca, B. L., Laupa Román, J. M., Guerra Bustios, P. C., Revilla, N. S., Tupayachi, R., Girardin, C. A. J., Doughty, C. E., and Malhi, Y.: Herbivory makes major contributions to ecosystem carbon and nutrient cycling in tropical forests, Ecol. Lett., 17, 324–332, https://doi.org/10.1111/ele.12233, 2014.
Meza-Canales, I. D., Meldau, S., Zavala, J. A., and Baldwin, I. T.: Herbivore perception decreases photosynthetic carbon assimilation and reduces stomatal conductance by engaging 12-oxo-phytodienoic acid, mitogen-activated protein kinase 4 and cytokinin perception, Plant Cell Environ., 40, 1039–1056, https://doi.org/10.1111/pce.12874, 2017.
Monsi, M. and Saeki, T.: Ueber den Lichtfaktor in den Planzengesellschaften und seine Bedeutung fuer die Stoffproduktion, Journal of Japanese Botany, 14, 22–52, 1953 (in German).
Nabity, P. D., Zavala, J. A., and DeLucia, E. H.: Indirect suppression of photosynthesis on individual leaves by arthropod herbivory, Annals of Botany, 103, 655–663, https://doi.org/10.1093/aob/mcn127, 2009.
Nykänen, H. and Koricheva, J.: Damage-induced changes in woody plants and their effects on insect herbivore performance: a meta-analysis, Oikos, 104, 247–268, 2004.
Pick, J. L., Nakagawa, S., and Noble, D. W. A.: Reproducible, flexible and high‐throughput data extraction from primary literature: The metaDigitise r package, Methods Ecol. Evol., 10, 426–431, https://doi.org/10.1111/2041-210X.13118, 2019.
Pilipović, A., Drekić, M., Stojnić, S., Nikolić, N., Trudić, B., Milović, M., Poljaković-Pajnik, L., Borišev, M., and Orlović, S.: Physiological responses of two pedunculate oak (Quercus robur L.) families to combined stress conditions – drought and herbivore attack, Šumarski list, 144, 582–583, https://doi.org/10.31298/sl.144.11-12.5, 2020.
Pincebourde, S. and Ngao, J.: The impact of phloem feeding insects on leaf ecophysiology varies with leaf age, Front. Plant Sci., 12, 625689, https://doi.org/10.3389/fpls.2021.625689, 2021.
Pustejovsky, J. E. and Rodgers, M. A.: Testing for funnel plot asymmetry of standardized mean differences, Res. Synth. Methods, 10, 57–71, https://doi.org/10.1002/jrsm.1332, 2019.
Quentin, A. G., Pinkard, E. A., Beadle, C. L., Wardlaw, T. J., O'Grady, A. P., Paterson, S., and Mohammed, C. L.: Do artificial and natural defoliation have similar effects on physiology of Eucalyptus globulus Labill. seedlings?, Ann. For. Sci., 67, 203–203, https://doi.org/10.1051/forest/2009096, 2010.
R Core Team: R: A language and environment for statistical computing, R Foundation for Statistical Computing, Vienna, Austria, https://www.R-project.org/ (last access: 19 November 2024), 2022.
Retuerto, R., Fernandez-Lema, B., Rodriguez-Roiloa, and Obeso, J. R.: Increased photosynthetic performance in holly trees infested by scale insects, Funct. Ecol., 18, 664–669, https://doi.org/10.1111/j.0269-8463.2004.00889.x, 2004.
Schäfer, K. V. R., Clark, K. L., Skowronski, N., and Hamerlynck, E. P.: Impact of insect defoliation on forest carbon balance as assessed with a canopy assimilation model, Glob. Change Biol., 16, 546–560, https://doi.org/10.1111/j.1365-2486.2009.02037.x, 2010.
Schaffer, B. and Mason, L. J.: Effects of scale insect herbivory and shading on net gas exchange and growth of a subtropical tree species (Guaiacum sanctum L.), Oecologia, 84, 468–473, https://doi.org/10.1007/BF00328162, 1990.
Schmitz, O. J., Raymond, P. A., Estes, J. A., Kurz, W. A., Holtgrieve, G. W., Ritchie, M. E., Schindler, D. E., Spivak, A. C., Wilson, R. W., Bradford, M. A., Christensen, V., Deegan, L., Smetacek, V., Vanni, M. J., and Wilmers, C. C.: Animating the carbon cycle, Ecosystems, 17, 344–359, https://doi.org/10.1007/s10021-013-9715-7, 2014.
Schwachtje, J. and Baldwin, I. T.: Why does herbivore attack reconfigure primary metabolism?, Plant Physiol., 146, 845–851, https://doi.org/10.1104/pp.107.112490, 2008.
Shestakov, A. L., Filippov, B. Yu., Zubrii, N. A., Klemola, T., Zezin, I., Zverev, V., Zvereva, E. L., and Kozlov, M. V.: Doubling of biomass production in European boreal forest trees by a four-year suppression of background insect herbivory, Forest Ecol. Manag., 462, 117992, https://doi.org/10.1016/j.foreco.2020.117992, 2020.
Snyder, K. A.: Night-time conductance in C3 and C4 species: do plants lose water at night?, J. Exp. Bot., 54, 861–865, https://doi.org/10.1093/jxb/erg082, 2003.
Stevens, M. T., Kruger, E. L., and Lindroth, R. L.: Variation in tolerance to herbivory is mediated by differences in biomass allocation in aspen, Funct. Ecol., 22, 40–47, https://doi.org/10.1111/j.1365-2435.2007.01356.x, 2008.
Stiling, P., Moon, D., Rossi, A., Hungate, B. A., and Drake, B.: Seeing the forest for the trees: long-term exposure to elevated CO2 increases some herbivore densities, Glob. Change Biol., 15, 1895–1902, https://doi.org/10.1111/j.1365-2486.2009.01902.x, 2009.
Strickland, M. S., Hawlena, D., Reese, A., Bradford, M. A., and Schmitz, O. J.: Trophic cascade alters ecosystem carbon exchange, P. Natl. Acad. Sci. USA, 110, 11035–11038, https://doi.org/10.1073/pnas.1305191110, 2013.
Thomas, M. V., Malhi, Y., Fenn, K. M., Fisher, J. B., Morecroft, M. D., Lloyd, C. R., Taylor, M. E., and McNeil, D. D.: Carbon dioxide fluxes over an ancient broadleaved deciduous woodland in southern England, Biogeosciences, 8, 1595–1613, https://doi.org/10.5194/bg-8-1595-2011, 2011.
Visakorpi, K.: Low-intensity insect herbivory could have large effects on ecosystem productivity through reduced canopy photosynthesis. R code and data, Version v1, Zenodo [data set/code], https://doi.org/10.5281/zenodo.13886133, 2024.
Visakorpi, K., Gripenberg, S., Malhi, Y., Bolas, C., Oliveras, I., Harris, N., Rifai, S., and Riutta, T.: Small-scale indirect plant responses to insect herbivory could have major impacts on canopy photosynthesis and isoprene emission, New Phytol., 220, 799–810, https://doi.org/10.1111/nph.15338, 2018.
Visakorpi, K., Riutta, T., Malhi, Y., Salminen, J.-P., Salinas, N., and Gripenberg, S.: Changes in oak (Quercus robur) photosynthesis after winter moth (Operophtera brumata) herbivory are not explained by changes in chemical or structural leaf traits, PLoS ONE, 15, e0228157, https://doi.org/10.1371/journal.pone.0228157, 2020.
Wagner, D., Wheeler, J. M., and Burr, S. J.: The leaf miner Phyllocnistis populiella negatively impacts water relations in aspen, Tree Physiol., 40, 580–590, https://doi.org/10.1093/treephys/tpz109, 2020.
Warrington, S., Cottam, D. A., and Whittaker, J. B.: Effects of insect damage on photosynthesis, transpiration and SO2 uptake by sycamore, Oecologia, 80, 136–139, https://doi.org/10.1007/BF00789943, 1989.
Whitehead, D., Griffin, K. L., Turnbull, M. H., Tissue, D. T., Engel, V. C., Brown, K. J., Schuster, W. S. F., and Walcroft, A. S.: Response of total night-time respiration to differences in total daily photosynthesis for leaves in a Quercus rubra L. canopy: implications for modelling canopy CO2 exchange, Glob. Change Biol., 10, 925–938, https://doi.org/10.1111/j.1529-8817.2003.00739.x, 2004.
Wickham, H.: Ggplot2: elegant graphics for data analysis, Springer, New York, 212 pp., https://doi.org/10.32614/CRAN.package.ggplot2, 2009.
Wilmers, C. C. and Schmitz, O. J.: Effects of gray wolf-induced trophic cascades on ecosystem carbon cycling, Ecosphere, 7, e01501, https://doi.org/10.1002/ecs2.1501, 2016.
Zangerl, A. R., Hamilton, J. G., Miller, T. J., Crofts, A. R., Oxborough, K., Berenbaum, M. R., and DeLucia, E. H.: Impact of folivory on photosynthesis is greater than the sum of its holes, P. Natl. Acad. Sci. USA, 99, 1088–1091, https://doi.org/10.1073/pnas.022647099, 2002.
Zimov, N. S., Zimov, S. A., Zimova, A. E., Zimova, G. M., Chuprynin, V. I., and Chapin III, F. S.: Carbon storage in permafrost and soils of the mammoth tundra-steppe biome: Role in the global carbon budget, Geophys. Res. Lett., 36, L02502, https://doi.org/10.1029/2008GL036332, 2009.
Zvereva, E. L., Zverev, V., and Kozlov, M. V.: Little strokes fell great oaks: minor but chronic herbivory substantially reduces birch growth, Oikos, 121, 2036–2043, https://doi.org/10.1111/j.1600-0706.2012.20688.x, 2012.